
Figure 1. Thought-sketch of conceptual steps that interconnect atoms at the lowest tier to bulk forms of materials at the highest tier of a hierarchical assembly passing through intermediate levels of complexity comprised of atom clusters to quantum confined nanoscale materials. Image copyright Todd Siler ArtNano Innovations.
I have been cogitating about the idea of a nanomaterials kaleidoscope, a type of combinatorial strategy for the design and synthesis of complex nanomatter from nanomaterials building blocks, a vision of a DNA of nanochemistry, which essentially mirrors the reality but not the details of the information content, complexity, growth, development and natural beauty underpinning basic and applied genomic research.
My thinking about a nanomaterials kaleidoscope was inspired by work on the human genome project, which began in 1989, and the recent emergence of research on a materials genome project in 2011, the former intended to create information databases to hasten the pace of discovery in biomedical research by mapping the human genome for the benefit of humanity and the latter to facilitate the more rapid transformation of innovative materials ideas into new products and processes in the marketplace.
Thinking about the biological world, a gene denotes a specific sequence of nucleotide bases in a strand of DNA that encode proteins and define function in an organism. The gene is the biomolecular carrier of heredity, the biochemical control system of life. In this context, it is worth mentioning that the DNA genome project was only possible after DNA was recognized as being the central molecule that leads to protein generation and cell/life function. It is a way of working back from molecularly complex to molecularly simple, call it ‘simplexity’. In a similar vein, I envision the development of a nanomaterials genome, namely the inherited behavior of complex nanomatter from its constituent nanomaterials building blocks, has the potential to serve the nanoscience community with a similar opportunity to speed up the development continuum of nanomaterials through the steps of discovery, structure determination and property optimization, functionality elucidation, system design and integration, certification, manufacturing to deployment of nanomaterials enabled technologies.
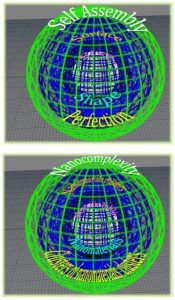
Figure 2. Nested sphere illustrations that portray the connection between the basic concepts of nanochemistry, size, shape, surface, perfection (top) and how nanocomplexity emerges from the hierarchical assembly of nanomaterials building blocks (bottom) leading at the topmost tier of the assembly to the far-reaching notion of a nanomaterials genome. Images courtesy Dr. Wendong Wang.
By a nanomaterials kaleidoscope I imply the formation of complex nanomatter that evolves from the pre-programmed assembly of nanoscale pieces of inorganic matter that in their bulk form behave for example as metals, semiconductors or insulators and when reduced to the nanometer scale adopt distinctive properties that follow the scaling rules of quantum confinement.
My thinking begins with Mendeleev who organized the elements that were known in his time in the form of a two-dimensional periodic table that provided a palette of atomic building blocks for making materials. In this same vein I foresee a multidimensional periodic table of nanomaterials construction units organized in terms of composition, size, shape, surface and degree of perfection from which evolves complex nanomatter with information encoded in a genomic format.
In this perspective, I see the conceptual steps that interconnect materials to nanomaterials to atom clusters and atoms not just spiraling upwards and downwards implicit in the curious ‘nexus’ that appears in the middle of the general thought-sketch displayed in Figure 1 but pictured as intertwined by nature, like the two helical ladders that are integrated by the four nucleotides that make up DNA. In this picture, the analogue of the four basic construction units that encode information into DNA (adenine, guanine, cytosine, thymine, AGCT) are the building blocks of size, shape, surface and perfection of nanomaterials, which through long range colloidal forces between building blocks are driven to assemble into multidimensional complex pre-programmed forms of nanomaterials clusters and clusters of nanomaterials clusters, with embedded information rich in content, portending and enabling advanced materials and biomedical technologies. There is of course a problem with this picture related to the fact that the key of the genome and of chemical function displayed by proteins and living organisms is the fact that the morphology is encoded with information and this information is written, to an extent, in the morphology. What we are currently capable of doing with nanomaterials is to make either periodic patterns in superlattice films and supercrystals, which only contain the structure and property information of the unit cell or amorphous assemblies, which contain less usable information as it is not non-predictable and/or non-deterministic.
What do I mean by the information encoded in a nanomaterials kaleidoscope? It is the chemical, and physical properties contained within and imprinted upon the surface of the nanomaterial building blocks that are integrated hierarchically, tier by tier, to higher and higher levels of complexity in an assembly to create new properties, new functions, new identities and new uses, which transcend those of the individual construction modules, Figure 2. The challenge of course is how to gain access to the ‘information encoded in complex nanomatter’ and how to utilize this information in a purposeful way to facilitate the long and arduous road from nanomaterials discovery to nanotechnology. This will necessitate adopting a ‘reverse engineering’ experimental approach complemented by a computational materials modeling strategy to elucidate how each particular choice and arrangement of constituent nanomaterials building blocks cooperate to provide the emergent properties, form, function and utility of a nanomaterials assembly.
The size (1-100 nm), shape (e.g., sphere, cube, octahedron, prism, tetrapod, rod, tube, sheet), surface (e.g., structure, charge, ligand) and degree of perfection (e.g., intrinsic variations in size, shape and surface, and intentional or unintentional presence of dopants, defects and impurities) of these modules carry embedded information on functionality, such as their optical, electrical, magnetic, mechanical and chemical behavior. It is the particular geometrical and spatial arrangement of these construction units driven by the forces of self-assembly that access increasing levels of nanomatter complexity, which we see through a ‘nanomaterials kaleidoscope’ and imagine as a ‘nanomaterials gene’ with materials character traits, inherited from its nanomaterials building-block code, an instruction blueprint that ultimately affords it with utility in advanced materials and biomedical technologies. Thinking about nanochemistry in this way, through a type of combinatorial nanochemistry, does add a number of variables to our nanomaterials design portfolio and those variables can be entangled in nontrivial ways to build a library of nanomaterials where changing each of the parameters allows one to be able to predict the output property. This is an important capability but the analogy with DNA is limited and should be treated with care. Information is useful when it is processed in a complex way through an algorithm which is what the DNA represents and we have a long way to go before we can do this with nanomaterials.
I wonder if a nanomaterials kaleidoscope can be an exemplar for thinking about complex nanomatter built from bricks-and-mortar where the bricks are nanoscale forms of metal, semiconductor or insulator materials with pre-determined sizes and shapes, and the mortar is afforded by their surfaces, which through colloidal interactions directs and assembles the bricks into aggregates traversing multiple length scales to create increasing degrees of nanomatter complexity at rising levels of the assembly.

Figure 3. Graphical representation of a nested-sphere concept of a multi-dimensional periodic table of nanomaterials depicting some of the infinite number of possible combinations and permutations of nanomaterials elemental compositions, sizes, shapes, surfaces and degree of perfection arranged on the points where longitude and latitude lines cross on the exterior surface of different spherical shells starting from the inside shell and moving outwards. Image courtesy Dr. Wendong Wang.
In this model of generating nanomatter complexity, the key components of the growth process make use of charge and sterically stabilized nanometer scale building blocks, between which operate colloidal repulsive, attractive and compaction forces for stabilization and entropic forces, capillary interactions, electric and magnetic fields for assembly into different forms of complex nanomatter.
In this model of generating nanomatter complexity, the key components of the growth process make use of charge and sterically stabilized nanometer scale building blocks, between which operate colloidal repulsive, attractive and compaction forces for stabilization and entropic forces, capillary interactions, electric and magnetic fields for assembly into different forms of complex nanomatter.
What do I mean by a ‘periodic table of nanomaterials’? While Mendeleev’s periodic table is a two-dimensional planar arrangement that organizes the chemical elements according to increasing atomic numbers, electronic configurations and trends in chemical behavior, I imagine a ‘periodic table of nanomaterials’ to be a multidimensional organizational scheme. One way I visualize this scheme is in the format of nested spheres, where the grid points defined by the intersections of adjacent longitudinal and latitudinal lines on every shell, from the center-out, depict the infinite number of possibilities (i.e., encoded information content) that can be amassed through combinations and permutations of composition, size, shape, surface, perfection and self-assembly to provide complex nanomatter with functionality and utility, Figure 3.
To amplify upon this idea, let the innermost sphere represent the elements ordered by atomic weight at grid points with a format that maintains the familiar periodic table structure of blocks, rows and columns. This sphere-shaped periodic table of the elements is surrounded by a sphere that represents all possible nanomaterials size variations for different compositions. Running north to south on a particular longitudinal line one tabulates at each grid point nanomaterials with monotonically diminishing size with a specific elemental composition. Each longitudinal line on this sphere depicts a nanomaterials element composition. The same model applies to the surrounding spheres which consecutively represent variations of nanomaterials shape, surface and perfection, thereby graphically depicting the infinite number of possible combinations of nanomaterials compositions, sizes, shapes, surfaces and perfection starting from the inside shell and moving outwards in the spherical nest, Figure 3.

Figure 4. Telescope representation of a multi-dimensional periodic table of nanomaterials where each cylindrical component of the telescope, from smallest to largest, when rotated with respect to one another can depict a kaleidoscope of combinations of nanomaterials composition, size, shape, surface, degree of perfection and self-assembly and hence the infinite number of nanotechnology opportunities.
Taken together, these possibilities can be seen to embrace concepts of nanochemistry for the synthesis of nanomaterials and express the idea of nanocomplexity through hierarchical assembly of nanomaterials to nanomaterials clusters, clusters of nanomaterials clusters, ultimately to provide complex nanomatter and its application opportunities in advanced materials and biomedical technologies. Nested shells of exemplary nanomaterials sizes, shapes, surfaces and perfection are illustrated in the diagram to bring out the essence of the graphical rendering of nets of nanocomplexity.
Another way of representing this same concept is to deconstruct the nested spheres into their constituent smaller spherical forms, in order to more clearly reveal the interior architecture and design of each, inspired by the Russian Matryoshka nested doll. Perhaps the separated spherical shells are not necessarily identical in size, shape and surface but rather vary slightly to highlight the possibility that they may be subtly asymmetrical and irregular, meaning, they’re not perfect in all aspects of the nano concepts and design principles rendered.
Yet another way to visualize a multidimensional periodic table of nanomaterials is in the form of a telescope of concentric coaxial cylinders intended to provide a kaleidoscope of nanomaterials compositions, sizes, shapes and surfaces with varying degrees of perfection that can be hierarchically assembled into nanomaterials clusters and clusters of nanomaterials clusters at increasing tiers of nanocomplexity, as sketched in Figure 4. In the tradition of Sir David Brewster, the Scottish inventor of the kaleidoscope in 1817, named from the Ancient Greek (kalos: beautiful, eidos: form, skopeo: to look) I would argue that this representation of a multidimensional periodic table of nanomaterials in the configuration of a ‘nano kaleidoscope’ makes it the ‘observer of beautiful forms’ through its multiple dimensions, its boundless forms and its cornucopia of opportunities for facilitating the transformation of creative ideas to innovative nanotechnologies.
Some examples of different kinds of complex nanomatter assembled from simple nanomaterial building blocks, that can be considered to take us closer to the recognition of nanocomplexity and a nanomaterials kaleidoscopic way of thinking, include the following:
- Nanocrystal clusters and nanocrystal crystals
- Nanorod supercrystals
- Nanotetrapod networks and lattices
- Stimulus responsive assembly and disassembly of nanocrystal crystals
- Step-growth polymerization of nanorod chains
- Nanocrystal Langmuir Blodgett film
- Binary nanocrystal superlattice films
- DNA programmable assembly of nanocrystal clusters, crystals and films
- Bio-hybrid crystals of nanoparticles and protein cages
- Nanocrystal rods and opals
- Nanocrystal electrochromic Bragg mirror
- Nanocrystal metamaterials
Take the case of end-functionalized gold nanorod chains where the component gold nanorod building blocks in the chain are pretty monodisperse and regular in shape. Here I contend the encoded information is encapsulated in the nanorod size and shape, nanorod chain aggregation number and nanorod chain conformation, which can be either cis- or trans- because of the faceted structure of the tips of the nanorods. These gold nanorod chains therefore contain an immense package of encoded information manifest not just in their overall structure but also in their plasmonic properties. I think this example and the others referenced above in my list of complex nanomatter are similarly crammed with vast amounts of encoded information beyond just the collection of nanomaterials building blocks but together with the properties of the whole, a kind of self programmed complex nanomatter.
On a point of clarification, mapping the information content of the genetic code of biological organisms involves DNA sequencing of the chromosomes via a deconstruction analytical protocol. By contrast the nanomaterials kaleidoscope I have in mind is intended as a blueprint to guide the formation of complex nanomatter by design rather than chance. In this framework, colloidal forces between nanoscale building blocks that are pre-programmed with size, shape and surface information, drive them into higher level architectures, such as nanocrystal chains, crystals, superlattices, photonic crystals and metamaterials as mentioned above. Here it is interesting to draw an analogy between DNA Origami and nanomaterials engineering, where I see the pre-programmed nucleotide sequences encoded in the linear strands of DNA and their sticky ends designed to enable the assembly of targeted complex DNA topologies, as mirroring the action of the size, shape and surface functional group information built into nanomaterials with respect to their ability to direct their assembly into more complex forms of nanomatter.
A nanomaterials kaleidoscope that captures nanomaterials assembly principles and maps the information encoded in the above mentioned assemblies can serve to embrace in an appealing form the creation of complex nanomatter from simple nanomaterial constituents with property-function relations that portend a profusion of nanotechnology applications.
As a case in point consider how the dynamics of nanomaterials exemplified by dissociative-associative exchange of nanocrystal surface ligands and reversible construction-deconstruction of nanocrystal clusters in response to changes in their environment raises the possibility of a combinatoric nanochemistry optimization strategy. This approach uses a dynamic library of reversibly exchanging nanocrystals evolving to an adaptive nanochemistry through dynamical nanocrystal variation and selection, a ‘Darwinian nanochemistry’ for self-sorting and self-discovery of the best performing complex nanomatter for a targeted purpose.
In practice, a problem with taking nanochemistry to this high level of structural sophistication is the challenge of achieving the control and uniformity of which biology makes good use. For example sp3 carbon reliably has four neighbors, not three or five, at the corners of a tetrahedron because of quantum mechanical rules. And all those biological macromolecules, whose wonderful asymmetry enables all sorts of lock and key interactions, cranked out from the same gene are identical in primary structure. These atomic-level controls are used far up the hierarchical ladder in biology. Can we ever mirror this level of sophistication through nanochemistry knowing a population of nanomaterials is usually a poly-dispersion, albeit with a narrow polydispersity index even after the application of size separation methods like size exclusion chromatography, density gradient ultracentrifugation or size selective precipitation?
In this same vein, the concept of valence in chemistry, that is the number of bonds an atom can form with the same or other atoms, is difficult to realize with nanomaterials assembly. Advances in the gallant pursuit of ‘nanomaterials molecules’ with pre-determined geometry are slowly being realized with spatially-controlled and surface-functionalized (i.e., hybridized) nanomaterials, where chemical ‘patches’ on the surface of the nanomaterials direct the assembly of the nanomaterials amongst themselves or with other molecules and nanomaterials. Actually the lack of deterministic valence can be considered both a problem and a blessing, as one can construct many more complex clusters based on nanoclusters with different exposed facets. For example, an icosahedral nanocluster would have a valence of twenty and a tetrahedral nanocluster just four although the material these clusters are made out of can be the same.
Another issue is that in biology and chemistry, new forms of complex matter often exhibit new functions. An example of this is the allosteric enzyme with two or more binding sites, where a binding event at one cooperatively influences the other. The central dogma of nanochemistry is that size, shape and surface matters, but it is not yet clear that building more complex nanomatter will lead to new properties and new functionality. To do this, one must understand how to put nanomaterial building blocks together in a meaningful way so that cooperative functionality will be achieved in the assembled nanomaterial. Here the concept of the ‘nanomatter enzyme’ is perhaps something to think about, where tweaking one site of a cluster of nanomaterials clusters causes a response at another site, such as loss or exchange of a capping ligand or building block or more dramatically induces the cluster assembly to reconstruct or deconstruct.
We have a long way to go to develop this structural richness and fidelity in nanochemistry before we even get close to the problem of mimicking the central dogma of biology and achieving the complexity and degree of perfection of the genes of living organisms. But we can start with building less than perfect hierarchical structures made of nanomaterials with properties and functionality that do not demand such a high degree of structural perfection and still get us a long way towards reaching certain goals. Indeed making such defect tolerant complex nanomatter is what most practitioners of the field of nanochemistry currently do all the time.
The ability to exploit variations in the nature and degree of perfection of a hierarchical architecture to generate complex nanomatter portends cross-disciplinary potential to improve the performance of a myriad of advanced materials technologies that utilize passive and active electronic, optical, photonic, magnetic, mechanical and chemical components. The approach also provides opportunities for enabling radically new solutions for a sustainable future in energy, climate, environment and human health.
There are so many extraordinarily impressive case histories in the recent literature that re-enforces this nanochemistry paradigm of nanocomplexity, which strengthens our notion of a kaleidoscopic way of thinking about the rapidly emerging nanomaterials world. While still in a nascent state-of-development, we look forward with anticipation to nanochemistry research that targets a universal scheme for organizing the information content of nanomaterials into a multidimensional compartmentalized form that brings forth periodic trends of the chemical and physical properties of nanomaterials and complex nanomatter made of nanomaterials assemblies. This scheme and these properties are imagined to be akin to Mendeleev’s periodic table of the elements that provides a rationalization of elemental properties such as electronic configurations, atomic radii, ionization potentials, electron affinities, electronegativities and cohesive energies. Armed with a multidimensional tabulation of nanomaterials and their assemblies, the ideas presented herein of mapping the myriad forms of complex nanomatter could become a reality.
In closing it is worth noting the idea of “messages encoded in complex nanomatter” is akin to Jean Marie Lehn’s “messages in supramolecular materials” – the challenge of any attempts to develop a genomic rather than a kaleidoscopic approach to nanochemistry as well as supramolecular chemistry is how to get access to that information and how to use it in a purposeful manner. A reverse engineering approach of deconstructing complex nanomatter into its information (property) carrying nanomaterials building units is perhaps the best way forward to make the nanomaterials kaleidoscope project scientifically and technologically worthwhile.