Imaging a black hole does not just happen. It is the result of a long scientific but also sociological process: the pioneering work of a few and the collective effort of many, understanding the basic physics, long term visions, sudden revelations, technological game changers, continuously growing incremental insights, and in the end, also some luck.
Witnessing how such a process unfolds from the beginning was an interesting experience for me. Here, I try to tell the story from my personal perspective and mention a few of the lessons I learned along the way.
First forays
As a student, I was drawn into physics by the fundamental questions about space, time, and matter that it addressed. Particle physics was at its peak and I was impressed by the large collaborations and the success of the Standard Model in ruling our world view. However, it was also clear that particle physics was getting too big. The ability to make an impact was getting more difficult and the next big thing was perhaps decades away.
But there was still one major, unsolved question: What is the true nature of gravity? After all, it was the last force resisting assimilation into the Standard Model of physics. Was it a force at all? How would it relate to quantum physics? One of the most mysterious objects that encapsulated all these questions seemed to be black holes. Did they really exist in nature?
When I started with my Ph.D., I witnessed an ambiguous situation. Everybody was talking about black holes, but they were still seen as exotic and unproven — ruling paradigm, but not a physical reality (yet). Many skeptics demanded more proof.

So, when new observations of Sagittarius A* (Sgr A*) — the hypothesized supermassive black hole at the center of our galaxy — came about in the 1990’s, it seemed clear that something exciting was in the air and as new observational opportunities abounded, the hunt for the supermassive black hole in our galaxy was on!
Modeling Sgr A* — if you know one black hole, you know them all
Surprisingly, there was no clear understanding of what the nature of the radio and sub mm-emission of Sgr A* were. A few months earlier we had investigated the accretion rate and luminosity of Sgr A*, i.e., how much matter was falling towards the black hole and how much energy was dissipated into radiation. We concluded that these properties were extremely low — we called it a “black hole on a starvation diet”.
We then wondered whether the radio emission of Sgr A* could be related to the jets — outflows of ionized matter emitted along the axis of rotation — seen in much more powerful quasars and radio galaxies, but at much lower levels. This would be unprecedented, but why not possible?
At the time, there was and still is a strange split in the community: Either one would work on jets from black holes, mainly related to radio astronomy and later, gamma-rays, or one would work on accretion disks, mainly working with optical or X-ray data. Communities in astronomy were traditionally determined by one’s favorite observing wavelength — that would literally determine your view of the universe and of individual objects.
But aren’t jets and disks not expressions of the same phenomenon and intimately linked? We later spoke a bit provocatively of the “jet-disk symbiosis” and our thought was that black holes should be simple.
From a grand perspective, their properties are only set by their mass — giving a length scale — and their accretion rate — setting the power scale. Spin and orientation of the black hole could modify the appearance further, but to a minor degree only.
So, if powerful black holes have a jet, why not feeble ones like our own? To get to the bottom of this, we postulated a fundamental coupling between the power of the inflow of materials feeding the black hole and the jet. We hypothesized that when we turn down the accretion rate — the rate at which black holes swallow matter — by a factor of one million, you get a jet power that is lowered by a factor of one million.
This model very naturally explains the characteristic spectrum of compact radio emissions found not only for the black hole at the center of the Milky Way, but for black holes in general. Indeed, we later hunted for this radio signature and found that it is ubiquitous in other low-power galactic nuclei.
When we applied this model to Sgr A*, we found that we only needed an accretion rate of 10-7 – 10-8 Msun (solar masses) per year to explain its faint radio emission and to reproduce its tiny size. This accretion rate is at least eight orders of magnitude lower than those derived for powerful quasars, and it is about four orders of magnitude lower than the average accretion rate for the Milky Way’s central black hole over the age of the universe. “Low”, however, still means that it consumes the mass of the Moon every few years.
Most excitingly, the model also suggested that the higher the frequency of the emission, the smaller and closer to the black hole’s event horizon it should be, meaning that it might be possible to see the darkness of this black hole as it swallows this radio light.
The scale we calculated in the 1990’s for Sgr A* was for a black hole mass ≈2×106 Msun and a distance from Earth of 8.5 kiloparsecs (kpc) where one kiloparsec, is 3,262 lightyears. Unfortunately, this was too small to be resolved by a measurement system called a very-long-baseline interferometry (VLBI) array in which a signal from an astronomical radio source, such as a quasar or black hole, is collected at multiple radio telescopes on Earth or in space.
At the time, the maximum possible resolution was not good enough. The smallest scale resolvable by an interferometer is given by the observing wavelength, which is fixed by the astrophysics of the black hole and the maximum telescope separation set by the size of the Earth — two parameters you cannot easily tune.
Disappointing, but the problem kept nagging at me.
A new vision
A few years later, I realized that we had overlooked an obvious effect. Searching for another paper in a book, I accidentally stumbled over a rather unknown paper by James Bardeen published in 1973, who had calculated the appearance of a putative rotating stellar mass black hole in front of a normal star. The black hole he showed was five times larger than the event horizon. Only then did it dawn on me that the strong gravitational lens effect would, of course, magnify the appearance of the event horizon in any image.
The basic equations for the light bending around a non-rotating black hole had already been calculated by David Hilbert in 1916. He had shown that light passing tangentially at a distance of √27 Rg would be asymptotically bent onto a circular photon orbit. Light rays coming any closer would end up in the event horizon while light rays passing further away can still escape.
Hence, the basic math for this problem had been found less than a year after Einstein formulated the theory of general relativity though its consequences took almost a century to sink in.
Shining light at a black hole and looking at it should therefore reveal a sharp divide between darkness and light with a circular shape of radius √27 Rg or only slightly less if the black hole was rotating. The effect, therefore, predicted a dark region in Sgr A* that is just resolvable by VLBI at wavelengths shortwards of 1.3 mm. The expected blurring of the image by interstellar scattering was expected to become subdominant at this wavelength.
Hence, it would actually be possible to start seeing the black hole with a realistic VLBI array at (sub)mm-waves!
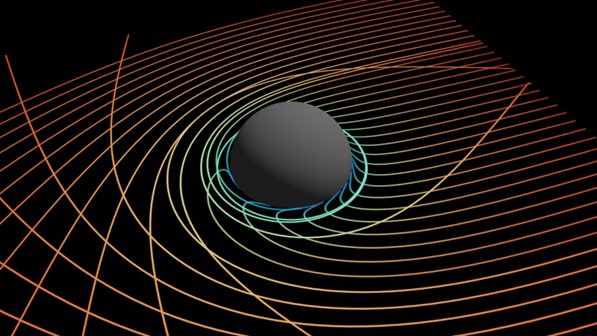
That thought was a life-changing revelation. Today, most libraries are gone and so are many old books. Nowadays, we preselect our literature with filters and bots or leave it to Google. I hope we still give luck a chance in science by occasionally turning an unexpected page somewhere.
Going further from that point needed more than just a vision. Some hard work had to still be done to make a convincing case. Millimeter-wave telescopes were around already, but some were threatened with closure, many still needed to be equipped, and new ones had to be built.
New opportunities appeared on the horizon with the planned Atacama Large Millimeter/submillimeter Array (ALMA) Telescope in Chile, the Large Millimeter Telescope (LMT) in Mexico, the upgrade of the Plateau de Bure Interferometer NOEMA, or later, the Green Land Telescope (GLT).
In the end, all would be used, but it took longer than expected.
Casting a shadow
To make the case clearer, we wanted to do a proper simulation of how light is bent by the black hole in Sgr A* and to make a more realistic theoretical image of it. After all, images capture imaginations.
We calculated the appearance of black holes for a wide range of generic astrophysical scenarios, where the black hole was embedded in an extended, transparent, glowing emission region, as expected for Sgr A*. We explored various spins as well as rotating, infalling, static, and outgoing flows and different radial emission profiles.
In the calculations, we broke with the standard picture of thin, opaque discs. In the standard picture one sees in popular magazines, the dark region is dominated by a gravitationally-lensed image of the hole in the accretion disk. Hence in this case, the key observable depends significantly on the astrophysics. For example, seen under an angle, the hole would appear egg-shaped since the inclined disc would obscure the black hole itself — it is hard to see a hole behind a wall.
However, in all our emission models, we always saw the close-to-circular dark region caused by the light bending. We called that region the “shadow” of the black hole, since one does not see the black hole itself, but rather the light it was blocking.
A simple picture to understand this is a wick in a burning candle, which leaves its dark imprint in the flame. Of course, in the case of the black hole one would not see any surface texture and the general relativistic black hole “wick” would appear larger than it actually is due to gravitational lensing.
For the sample models that we ultimately published (see below), we chose a red color scale, representing heat or molten iron. After all, we were showing radio emission that is invisible to the human eye as it comes from the extreme red part of the electromagnetic spectrum and is emitted by extremely hot gas. This was an artistic choice in the end, but it stuck.
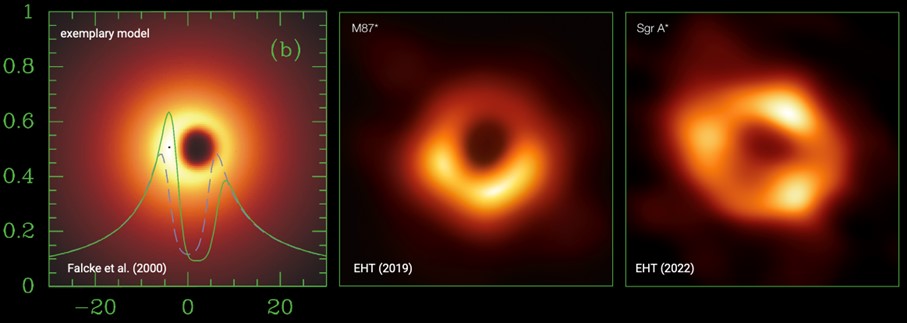
In our simulations, the shadow was often surrounded by a bright ring of emission, later called a photon ring. Sometimes only half or a quarter of the ring was clearly visible due to relativistic beaming. Emission from plasma that is moving towards the observer would be amplified, and plasma moving away, de-amplified. This leads to a characteristic crescent shape for a rotating emission region.
The figure below shows a few models that we calculated at the time for different generic astrophysical situations.
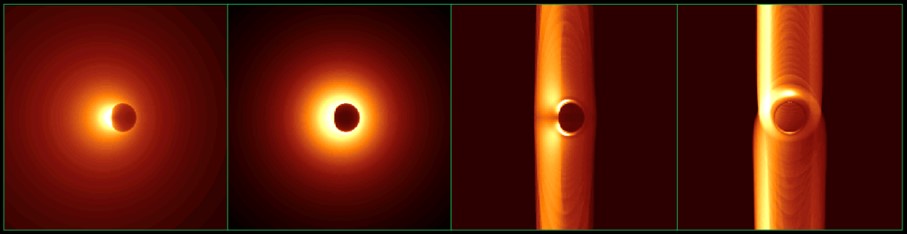
Reprinted with permission from the American Astronomical Society.
The circumference of the shadow itself is mathematically determined, and we concluded that the shadow is a very robust test to probe key characteristics of black holes and their spacetime.
Probing the waters and testing the model
Of course, probing the shadow in Sgr A* with telescopes would only work if the emission was indeed what we thought. We organized what was perhaps the first multi-wavelength campaign with multiple telescopes focused on Sgr A*.
Soon thereafter, the first prototype VLBI observation of Sgr A* at 1.3 mm was published by a small group at the MPIfR in Bonn using the two IRAM telescopes on Pico Veleta and the Plateau de Bure. Two telescopes are not enough to make an image, but at least a one-dimensional size could be measured. The inferred value was consistent with today’s measurements; however, the error bar was large and the conclusions unclear.
A few years later, we were able to get much better data at longer wavelengths and finally succeeded in deriving an intrinsic size of Sgr A*. It showed indeed that the higher you go in frequency, the closer you get to the black hole shadow. The actual source size was decreasing with wavelength. Extrapolating, one could show that event horizon scales would be reached at around 1mm wavelengths.
Building momentum
In 2004, we organized a special session at a conference in Green Bank dedicated to the 30th anniversary of the discovery of Sgr A*, where Geoff Bower, Shep Doeleman, and myself spoke. The discussion was geared towards preparing collaborative future experiments, and at the end of the session, there was strong support in the community for doing so.
“The goal of the consortium will be to not only image the shadow of the black hole once but to continue the experiment in the future. We want to turn Sgr A* into a precision laboratory to directly measure and image all kinds of general relativistic effects that cannot be seen anywhere else in the universe” — minutes from the March 3, 2006 telecon.
I was advocating a more formal collaboration since I had started to work in astroparticle physics and had seen how larger physics collaboration worked from the inside. However, it would take a little longer for it to take shape. Just at the time when (sub)mm-wave astronomy was entering a new phase, with ALMA as a major new project, we faced the danger of losing the smaller telescopes, necessary to do a VLBI experiment!
In 2008, Shepard Doeleman and collaborators organized a series of experiments with telescopes available in the US, which eventually succeeded with the first measurement at 1.3 mm with three telescope sites, including those two telescopes plus the Combined Array for Research in Millimetre-wave Astronomy (CARMA) in California. The result was more robust and had smaller uncertainties than the experiment from the 1990’s. Moreover, the mass measurements of Sgr A* had become more accurate and precise due to the measurements of stellar orbits around the object by the groups of Reinhard Genzel and Andrea M. Ghez.
The inferred mass had doubled and the inferred distance had shrunk compared to the 1990’s. This meant that the expected shadow had increased significantly and should be about 50 microarcsecond (µas) in diameter now. The new VLBI experiment then claimed 36+16-10 µas.
Clearly, there was event horizon-scale structure, and these experiments paved the way for the future Event Horizon Telescope — an array which linked together eight existing radio observatories across the planet to form a single “Earth-sized” virtual telescope.
In one of these famous conference coffee breaks, I talked with Doeleman and suggested choosing a more interesting name for the experiment, and we came up with “Event Horizon Telescope”. A name was born, but there was no consensus yet as to what it meant. Many meetings and telecons still had to follow.
Of course, there were also tensions and one could write an extra book about those. Not all of them were always constructive but in the long run, tension keeps you sharp and can help move things forward.
In 2013, I succeeded together with two colleagues in getting the €14M Synergy Grant “BlackHoleCam” of the European Research Council (ERC), which enabled us to fund not only hardware, but also data analysis, theory, and even pulsar research. This was the largest single grant ever given towards the Event Horizon Telescope, which also integrated all these components into one large project. Later, a major grant by the NSF was granted in the US with Doeleman as PI and many US colleagues contributing, and in 2015, the East Asian Observatory rescued the JCMT on Hawaii.
In 2014, the preliminary structure of the Event Horizon Telescope was decided on in a meeting at the Perimeter Institute in Canada and included 13 stakeholder institutes from Asia, Europe, and the Americas, all bringing in significant institutional resources and forming an initial board. The Event Horizon Telescope indeed became a comprehensive collaboration with the ambition to cover all aspects: Providing hardware, operating an array, calibrating and imaging data, analysis, and interpretation. This would later be reflected in the fact that the Event Horizon Telescope eventually published more than just a single image, but a comprehensive series of extensive papers covering a wide range of science.
The final Memorandum of Understanding (MoU) was only signed in 2017 after additional 50 interim board meetings, well after we had conducted our first full-scale groundbreaking experiment. The entire process reminded me of ancient nation building, where different tribes come together to form nations with an emerging legal and political system — fortunately, it was less bloody than in the old days, but not without stress and anxiety.
Feeling lucky
In 2017, we carried out our first, large-scale attempt at an experiment with eight telescopes on six different high sites. The crews were mixed, from different institutes. Our group had sent personnel to all mountains, except the South Pole, and met the other scientists and observers from other stakeholder groups in the Event Horizon Telescope.
I still remember looking at the marvelous blue sky at the IRAM 30m telescope at Pico Veleta, all the stories of weathered-out observations in the back of my mind. Millimeter waves are absorbed by water vapor in the atmosphere and you do not want to have a cloudy sky in between you and the universe. Amazingly, the weather was excellent around the globe during most of the ten-day observing run and all the equipment worked.
Fortunately, the universe also cooperated. I’ll never forget the moment when — one year later — my Ph.D. student, Sara Issaoun, showed the first data on M87* to me “hot off the press”. It was shouting at us: There are two black holes and you can see a shadow!
Repeatability and duplication became a guiding principle in the analysis. Every step was done by at least two independent groups using independent software. This principle of checks and balances through duplication took a while to solidify. However, competition is an equally powerful driver as the desire to get it right. Even in a collaboration that publishes with an alphabetically sorted author list, everybody wants to show that their algorithm is as good or even better than the other and duplication emerges almost naturally — if you do not suppress it actively.
In a way, this competitive collaboration was even reflected in how the first results were presented: Not in one central press conference, but in parallel ones in Brussels, Washington, Tokyo, Taipei, Santiago de Chile, and in additional satellite events. As a consequence, the release of the first set of papers and images became a truly global event.
For me, Brussels was a natural choice to recognize the contributions of the European taxpayer. It was an enormous privilege that I could present the image to the public after all these years, but it was also liberating after the intense months of collaborative work in secrecy. To me, this was the moment when the image became reality. It didn’t belong just to us anymore but became global property. We had taken the image with the world, we gave it back to the world, and the world embraced it like none of us ever expected.
More than four billion people were exposed to the image in the end. Of course, a large fraction of those people probably forgot about it within a few weeks. Behind the press release and the image was an entire book of scientific literature that defined an entirely new level of black hole science, opening many new avenues of research.
The final confirmation came with the publication of our second image: Finally, the image of Sgr A*, the black hole in the galactic center. It is 1,500 times smaller, 1,500 times closer, and 100,000 time less powerful than M87*, yet showed a very similar picture. Astrophysics and gravitational physics were giving the right answers — that is not a small thing.
That was important, because astrophysics has become a key pillar of today’s physics. If you want to derive physics from the extremes of the cosmos, you need to understand their astrophysics. Understanding the astrophysics surrounding black holes is as equally fundamental as understanding the inside of the atomic nucleus or the nature of quantum interactions.
With these observations, we have established the “shadow” as a fundamental and observable property of a black hole. Its size is accurately predicted by GR and scales linearly with mass. This is a very unusual property for any macroscopic object and this scaling is a distinct signature of black holes.
In objects made out of normal matter, like you and me, the mass roughly scales with the volume, i.e., with the third power of the radius, and not linearly. A tall boy of 2 years would be 92 cm tall and weigh 14.5 kg. At my age, he would be roughly six time heavier (5.8 times to be precise) but only twice as tall.
Growing like a black hole to my age and weight, he should be 5.30m tall! This difference in scaling we can now test with the Event Horizon Telescope alone over three orders of magnitude and over eight orders of magnitude if one includes gravitational waves where the error bars become almost irrelevant.
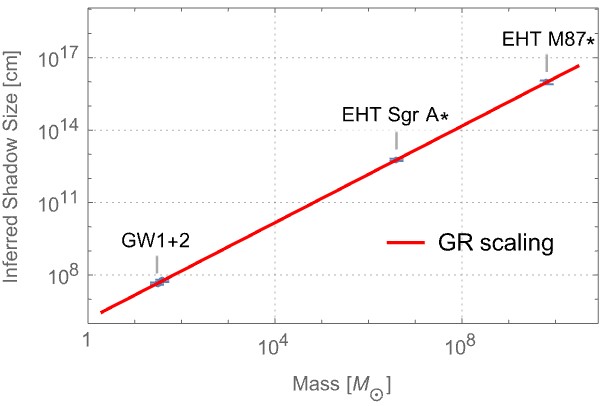
One other important realization is that we are in a regime of theory that can actually be tackled in a reasonable way. In fact, simulating a black hole is in principle easier than simulating our Sun. Of course, we still have much less detailed information about black holes than about the Sun, hence, there is less data to explain, but in principle this should be true, since black holes are described by much fewer parameters.
Some conclusions
We have witnessed an extraordinary period over the past three years. For the first time, we have seen images of black holes. There are only two objects, but they are representatives of very different types of galaxies and astrophysical conditions. We can now safely assume that the centers of galaxies do host supermassive black holes — from the first quasars to ordinary, run-of-the-mill galaxies like our own.
For the foreseeable future, Sgr A* and M87* will also be the last black holes we see as all the others are too small or too far away. However, in contrast to gravitational, wave-emitting black hole mergers, these black holes will stay around. We can observe them with ever better techniques and at all possible wavelengths.
With the Event Horizon Telescope, we can also study their temporal behaviors, maybe make a movie of their dynamics, and ever refine our models to be tested against observational data. This is what you expect from a good physics experiment.
In the future, we can turn towards space interferometry missions to image many more black holes and at much higher resolutions and image quality. This could bring at least another order of magnitude or more improvements in the measured shadow size and rule out a much larger range of parameter space of alternative models. We can also understand black hole astrophysics in detail, e.g., fully understand jet launching, measure black hole spin much more precisely, and verify such effects as spin energy extraction from black holes through magnetic fields, via the Blandford-Znajek effect.
Looking back at the past 25 years, it was a great privilege to witness how all of this unfolded. Getting to this point required enormous efforts by many scientists. Not one person, institute, or country alone could have realized this vision. Seeing how this collaboration emerged was almost as interesting as seeing how the science evolved.
Thanks to gravitational wave experiments, the Event Horizon Telescope, ESO’s near-infrared interferometer, Gravity, and pulsar observations we are now entering an era of experimental spacetime physics. We can test physical predictions that once seemed impossible when first calculated. We have not found a new theory of gravity yet, but we have learned more about the physics in a universe where spacetime is torn and twisted unlike anything we can ever create here on Earth.
This has also led to an avalanche of new tests of general relativity and new ideas that are being explored. We are now standing on solid ground when we use general relativity in the most extreme circumstances. Maybe it is time to dare to take one step further and try to come up with a new theory that goes further than Einstein.
In the end, experiments not only measure, but they also inspire. Hence, I hope that experiments like the Event Horizon Telescope will not only test old ideas, but also create room for new ones.
Featured image: The first image of Sgr A*, the supermassive black hole at the center of our galaxy. It’s the first direct visual evidence of the presence of this black hole captured by the Event Horizon Telescope. Credit: EHT Collaboration
This article was originally published in Natural Sciences, the interdisciplinary open access journal publishing top-tier research from biology, chemistry, physics and their interfaces. The article has been modified for this website version.
Follow Natural Sciences on Twitter @NatSci_journal